The developing toolkit of continuous directed evolution
Continuous directed evolution methods allow the key steps of evolution—gene diversification, selection, and replication—to proceed in the laboratory with minimal researcher intervention. As a result, continuous evolution can find solutions much more quickly than traditional discrete evolution methods. Continuous evolution also enables the exploration of longer and more numerous evolutionary trajectories, increasing the likelihood of accessing solutions that require many steps through sequence space and greatly facilitating the iterative refinement of selection conditions and targeted mutagenesis strategies. Here we review the historical advances that have expanded continuous evolution from its earliest days as an experimental curiosity to its present state as a powerful and surprisingly general strategy for generating tailor-made biomolecules, and discuss more recent improvements with an eye to the future.
This is a preview of subscription content, access via your institution
Access options
Access Nature and 54 other Nature Portfolio journals
Get Nature+, our best-value online-access subscription
cancel any time
Subscribe to this journal
Receive 12 print issues and online access
265,23 € per year
only 22,10 € per issue
Buy this article
- Purchase on SpringerLink
- Instant access to full article PDF
Prices may be subject to local taxes which are calculated during checkout
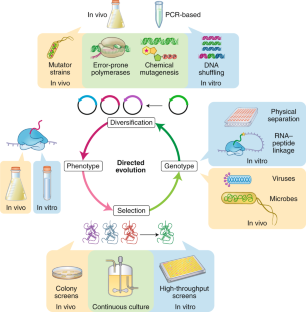
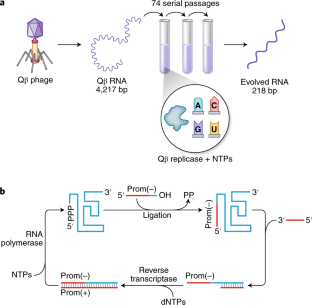
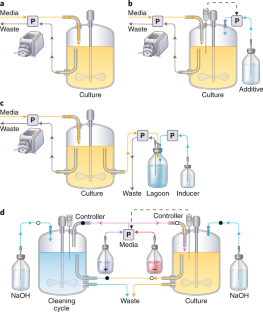
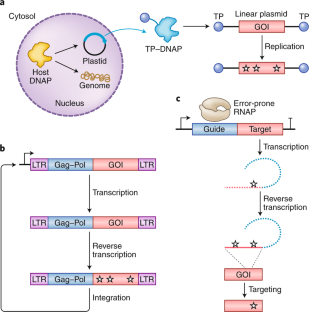
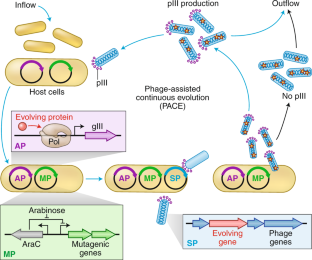
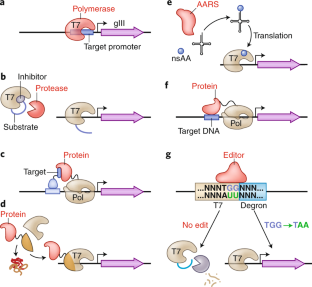
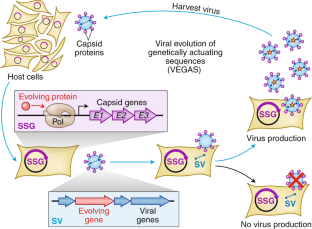
Similar content being viewed by others
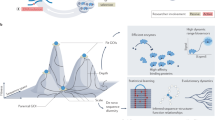
In vivo hypermutation and continuous evolution
Article 19 May 2022
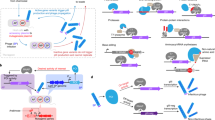
Phage-assisted continuous and non-continuous evolution
Article 16 November 2020
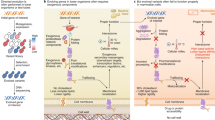
Directed evolution in mammalian cells
Article 07 April 2021
References
- Mills, D. R., Peterson, R. L. & Spiegelman, S. An extracellular Darwinian experiment with a self-duplicating nucleic acid molecule. Proc. Natl Acad. Sci. USA58, 217–224 (1967). ArticleCASPubMedGoogle Scholar
- Wright, M. C. & Joyce, G. F. Continuous in vitro evolution of catalytic function. Science276, 614–617 (1997). ArticleCASPubMedGoogle Scholar
- McGinness, K. E., Wright, M. C. & Joyce, G. F. Continuous in vitro evolution of a ribozyme that catalyzes three successive nucleotidyl addition reactions. Chem. Biol.9, 585–596 (2002). ArticleCASPubMedGoogle Scholar
- Breaker, R. R., Banerji, A. & Joyce, G. F. Continuous in vitro evolution of bacteriophage RNA polymerase promoters. Biochemistry33, 11980–11986 (1994). ArticleCASPubMedGoogle Scholar
- Kühne, H. & Joyce, G. F. Continuous in vitro evolution of ribozymes that operate under conditions of extreme pH. J. Mol. Evol.57, 292–298 (2003). ArticlePubMedCASGoogle Scholar
- Voytek, S. B. & Joyce, G. F. Emergence of a fast-reacting ribozyme that is capable of undergoing continuous evolution. Proc. Natl Acad. Sci. USA104, 15288–15293 (2007). ArticleCASPubMedGoogle Scholar
- Matsuura, T. & Yomo, T. In vitro evolution of proteins. J. Biosci. Bioeng.101, 449–456 (2006). ArticleCASPubMedGoogle Scholar
- Monod, J. La technique de culture continue: theorie et applications. Ann. Inst. Pasteur79, 390–410 (1950). CASGoogle Scholar
- Novick, A. & Szilard, L. Experiments with the Chemostat on spontaneous mutations of bacteria. Proc. Natl Acad. Sci. USA36, 708–719 (1950). ArticleCASPubMedGoogle Scholar
- Bryson, V. & Szybalski, W. Microbial selection. Science116, 45–51 (1952). ArticlePubMedGoogle Scholar
- Larsson, G., Enfors, S. O. & Pham, H. The pH-auxostat as a tool for studying microbial dynamics in continuous fermentation. Biotechnol. Bioeng.36, 224–232 (1990). ArticleCASPubMedGoogle Scholar
- Moser, H. The Dynamics of Bacterial Populations Maintained inthe Chemostat. (Washington D.C., Carnegie Institution of Washington, 1958).
- Bull, J. J. et al. Exceptional convergent evolution in a virus. Genetics147, 1497–1507 (1997). CASPubMedPubMed CentralGoogle Scholar
- Marlière, P. et al. Chemical evolution of a bacterium’s genome. Angew. Chem. Int. Ed. Engl.50, 7109–7114 (2011). ArticlePubMedCASGoogle Scholar
- Toprak, E. et al. Evolutionary paths to antibiotic resistance under dynamically sustained drug selection. Nat. Genet.44, 101–105 (2011). ArticlePubMedPubMed CentralCASGoogle Scholar
- Zamenhof, S. & Eichhorn, H. H. Study of microbial evolution through loss of biosynthetic functions: establishment of “defective” mutants. Nature216, 456–458 (1967). ArticleCASPubMedGoogle Scholar
- Lwoff, A. L’évolution Physiologique: Étude des Pertes de Fonctions chez les Microorganismes. (Paris, Hermann, 1944).
- Lenski, R. E. & Levin, B. R. Constraints on the coevolution of bacteria and virulent phage: a model, some experiments, and predictions for natural communities. Am. Nat.125, 585–602 (1985). ArticleGoogle Scholar
- Hillis, D. M., Bull, J. J., White, M. E., Badgett, M. R. & Molineux, I. J. Experimental phylogenetics: generation of a known phylogeny. Science255, 589–592 (1992). ArticleCASPubMedGoogle Scholar
- Greener, A., Callahan, M. & Jerpseth, B. An efficient random mutagenesis technique using an E. coli mutator strain. Mol. Biotechnol.7, 189–195 (1997). ArticleCASPubMedGoogle Scholar
- Chou, H. H. & Keasling, J. D. Programming adaptive control to evolve increased metabolite production. Nat. Commun.4, 2595 (2013). ArticlePubMedCASGoogle Scholar
- Pham, H. L. et al. Engineering a riboswitch-based genetic platform for the self-directed evolution of acid-tolerant phenotypes. Nat. Commun.8, 411 (2017). ArticlePubMedPubMed CentralCASGoogle Scholar
- Badran, A. H. & Liu, D. R. Development of potent in vivo mutagenesis plasmids with broad mutational spectra. Nat. Commun.6, 8425 (2015). ArticleCASPubMedPubMed CentralGoogle Scholar
- Wang, H. H. et al. Programming cells by multiplex genome engineering and accelerated evolution. Nature460, 894–898 (2009). ArticleCASPubMedPubMed CentralGoogle Scholar
- Mundhada, H. et al. Increased production of l-serine in Escherichia coli through adaptive laboratory evolution. Metab. Eng.39, 141–150 (2017). ArticleCASPubMedGoogle Scholar
- Amiram, M. et al. Evolution of translation machinery in recoded bacteria enables multi-site incorporation of nonstandard amino acids. Nat. Biotechnol.33, 1272–1279 (2015). ArticleCASPubMedPubMed CentralGoogle Scholar
- Martin, R. W. et al. Cell-free protein synthesis from genomically recoded bacteria enables multisite incorporation of noncanonical amino acids. Nat. Commun.9, 1203 (2018). ArticlePubMedPubMed CentralCASGoogle Scholar
- Barbieri, E. M., Muir, P., Akhuetie-Oni, B. O., Yellman, C. M. & Isaacs, F. J. Precise editing at DNA replication forks enables multiplex genome engineering in eukaryotes. Cell171, 1453–1467.e13 (2017). ArticleCASPubMedPubMed CentralGoogle Scholar
- Garst, A. D. et al. Genome-wide mapping of mutations at single-nucleotide resolution for protein, metabolic and genome engineering. Nat. Biotechnol.35, 48–55 (2017). ArticleCASPubMedGoogle Scholar
- Yano, T., Oue, S. & Kagamiyama, H. Directed evolution of an aspartate aminotransferase with new substrate specificities. Proc. Natl Acad. Sci. USA95, 5511–5515 (1998). ArticleCASPubMedGoogle Scholar
- Ravikumar, A., Arzumanyan, G. A., Obadi, M. K. A., Javanpour, A. A. & Liu, C. C. Scalable, continuous evolution of genes at mutation rates above genomic error thresholds. Cell175, 1957.e13 (2018).
- Fabret, C. et al. Efficient gene targeted random mutagenesis in genetically stable Escherichia coli strains. Nucleic Acids Res.28, E95 (2000). ArticleCASPubMedPubMed CentralGoogle Scholar
- Camps, M., Naukkarinen, J., Johnson, B. P. & Loeb, L. A. Targeted gene evolution in Escherichia coli using a highly error-prone DNA polymerase I. Proc. Natl Acad. Sci. USA100, 9727–9732 (2003). ArticleCASPubMedGoogle Scholar
- Ravikumar, A., Arrieta, A. & Liu, C. C. An orthogonal DNA replication system in yeast. Nat. Chem. Biol.10, 175–177 (2014). ArticleCASPubMedGoogle Scholar
- Crook, N. et al. In vivo continuous evolution of genes and pathways in yeast. Nat. Commun.7, 13051 (2016). ArticleCASPubMedPubMed CentralGoogle Scholar
- Simon, A. J., Morrow, B. R. & Ellington, A. D. Retroelement-based genome editing and evolution. ACS Synth. Biol.7, 2600–2611 (2018). ArticleCASPubMedGoogle Scholar
- Romanini, D. W., Peralta-Yahya, P., Mondol, V. & Cornish, V. W. A heritable recombination system for synthetic Darwinian evolution in yeast. ACS Synth. Biol.1, 602–609 (2012). ArticleCASPubMedPubMed CentralGoogle Scholar
- Finney-Manchester, S. P. & Maheshri, N. Harnessing mutagenic homologous recombination for targeted mutagenesis in vivo by TaGTEAM. Nucleic Acids Res.41, e99 (2013). ArticleCASPubMedPubMed CentralGoogle Scholar
- Moore, C. L., Papa, L. J. III & Shoulders, M. D. A processive protein chimera introduces mutations across defined DNA regions in vivo. J. Am. Chem. Soc.140, 11560–11564 (2018). ArticleCASPubMedPubMed CentralGoogle Scholar
- Hess, G. T. et al. Directed evolution using dCas9-targeted somatic hypermutation in mammalian cells. Nat. Methods13, 1036–1042 (2016). ArticleCASPubMedPubMed CentralGoogle Scholar
- Halperin, S. O. et al. CRISPR-guided DNA polymerases enable diversification of all nucleotides in a tunable window. Nature560, 248–252 (2018). ArticleCASPubMedGoogle Scholar
- Esvelt, K. M., Carlson, J. C. & Liu, D. R. A system for the continuous directed evolution of biomolecules. Nature472, 499–503 (2011). ArticleCASPubMedPubMed CentralGoogle Scholar
- Smeal, S. W., Schmitt, M. A., Pereira, R. R., Prasad, A. & Fisk, J. D. Simulation of the M13 life cycle I: assembly of a genetically-structured deterministic chemical kinetic simulation. Virology500, 259–274 (2017). ArticleCASPubMedGoogle Scholar
- Carlson, J. C., Badran, A. H., Guggiana-Nilo, D. A. & Liu, D. R. Negative selection and stringency modulation in phage-assisted continuous evolution. Nat. Chem. Biol.10, 216–222 (2014). ArticleCASPubMedPubMed CentralGoogle Scholar
- Dickinson, B. C., Packer, M. S., Badran, A. H. & Liu, D. R. A system for the continuous directed evolution of proteases rapidly reveals drug-resistance mutations. Nat. Commun.5, 5352 (2014). ArticleCASPubMedPubMed CentralGoogle Scholar
- Packer, M. S., Rees, H. A. & Liu, D. R. Phage-assisted continuous evolution of proteases with altered substrate specificity. Nat. Commun.8, 956 (2017). ArticlePubMedPubMed CentralCASGoogle Scholar
- Badran, A. H. et al. Continuous evolution of Bacillus thuringiensis toxins overcomes insect resistance. Nature533, 58–63 (2016). ArticleCASPubMedPubMed CentralGoogle Scholar
- Wang, T., Badran, A. H., Huang, T. P. & Liu, D. R. Continuous directed evolution of proteins with improved soluble expression. Nat. Chem. Biol.14, 972–980 (2018). ArticleCASPubMedPubMed CentralGoogle Scholar
- Bryson, D. I. et al. Continuous directed evolution of aminoacyl-tRNA synthetases. Nat. Chem. Biol.13, 1253–1260 (2017). ArticleCASPubMedPubMed CentralGoogle Scholar
- Hubbard, B. P. et al. Continuous directed evolution of DNA-binding proteins to improve TALEN specificity. Nat. Methods12, 939–942 (2015). ArticleCASPubMedPubMed CentralGoogle Scholar
- Hu, J. H. et al. Evolved Cas9 variants with broad PAM compatibility and high DNA specificity. Nature556, 57–63 (2018). ArticleCASPubMedPubMed CentralGoogle Scholar
- Miller, S.M. et al. Continuous evolution of SpCas9 variants compatible with non-G PAMs. Nat. Biotechnol. (2020).
- Thuronyi, B.W. et al. Continuous evolution of base editors with expanded target compatibility and improved activity. Nat. Biotechnol.37, 1070–1079 (2019). ArticleCASPubMedPubMed CentralGoogle Scholar
- Berman, C. M. et al. An adaptable platform for directed evolution in human cells. J. Am. Chem. Soc.140, 18093–18103 (2018). ArticleCASPubMedPubMed CentralGoogle Scholar
- English, J. G. et al. VEGAS as a platform for facile directed evolution in mammalian cells. Cell178, 748–761.e7 (2019). ArticleCASPubMedGoogle Scholar
- Butterfield, G. L. et al. Evolution of a designed protein assembly encapsulating its own RNA genome. Nature552, 415–420 (2017). ArticleCASPubMedPubMed CentralGoogle Scholar
- Voigt, C. A., Martinez, C., Wang, Z. G., Mayo, S. L. & Arnold, F. H. Protein building blocks preserved by recombination. Nat. Struct. Biol.9, 553–558 (2002). CASPubMedGoogle Scholar
- Wu, Z., Kan, S. B. J., Lewis, R. D., Wittmann, B. J. & Arnold, F. H. Machine learning-assisted directed protein evolution with combinatorial libraries. Proc. Natl Acad. Sci. USA116, 8852–8858 (2019). ArticleCASPubMedGoogle Scholar
- Wong, J. T. Membership mutation of the genetic code: loss of fitness by tryptophan. Proc. Natl Acad. Sci. USA80, 6303–6306 (1983). ArticleCASPubMedGoogle Scholar
- Wang, L. & Schultz, P. G. A general approach for the generation of orthogonal tRNAs. Chem. Biol.8, 883–890 (2001). ArticleCASPubMedGoogle Scholar
- Monk, J. W. et al. Rapid and inexpensive evaluation of nonstandard amino acid incorporation in Escherichia coli. ACS Synth. Biol.6, 45–54 (2017). ArticleCASPubMedGoogle Scholar
- Hammerling, M. J. et al. Bacteriophages use an expanded genetic code on evolutionary paths to higher fitness. Nat. Chem. Biol.10, 178–180 (2014). ArticleCASPubMedPubMed CentralGoogle Scholar
- Drienovská, I., Mayer, C., Dulson, C. & Roelfes, G. A designer enzyme for hydrazone and oxime formation featuring an unnatural catalytic aniline residue. Nat. Chem.10, 946–952 (2018). ArticlePubMedCASGoogle Scholar
- Mayer, C., Dulson, C., Reddem, E., Thunnissen, A. W. H. & Roelfes, G. Directed evolution of a designer enzyme featuring an unnatural catalytic amino acid. Angew. Chem. Int. Ed. Engl.58, 2083–2087 (2019). ArticleCASGoogle Scholar
- Kanigowska, P., Shen, Y., Zheng, Y., Rosser, S. & Cai, Y. Smart DNA fabrication using sound waves: applying acoustic dispensing technologies to synthetic biology. J. Lab. Autom.21, 49–56 (2016). ArticleCASPubMedPubMed CentralGoogle Scholar
- Terekhov, S. S. et al. Microfluidic droplet platform for ultrahigh-throughput single-cell screening of biodiversity. Proc. Natl Acad. Sci. USA114, 2550–2555 (2017). ArticleCASPubMedGoogle Scholar
- Wong, B. G., Mancuso, C. P., Kiriakov, S., Bashor, C. J. & Khalil, A. S. Precise, automated control of conditions for high-throughput growth of yeast and bacteria with eVOLVER. Nat. Biotechnol.36, 614–623 (2018). ArticleCASPubMedPubMed CentralGoogle Scholar
- Callens, C. et al. A multiplex culture system for the long-term growth of fission yeast cells. Yeast34, 343–355 (2017). ArticleCASPubMedPubMed CentralGoogle Scholar
- Takahashi, C. N., Miller, A. W., Ekness, F., Dunham, M. J. & Klavins, E. A low cost, customizable turbidostat for use in synthetic circuit characterization. ACS Synth. Biol.4, 32–38 (2015). ArticleCASPubMedGoogle Scholar
Acknowledgements
D.R.L. gratefully acknowledges support from NIH U01 AI142756 (D.R.L.), RM1 HG009490 (D.R.L.), R01 EB022376 (D.R.L.), and R35 GM118062 (D.R.L.); and HHMI (D.R.L.). We thank A. Badran and K. Zhao for their helpful comments.
Author information
Authors and Affiliations
- Merkin Institute of Transformative Technologies in Healthcare, Broad Institute of MIT and Harvard, Cambridge, MA, USA Mary S. Morrison, Christopher J. Podracky & David R. Liu
- Howard Hughes Medical Institute, Harvard University, Cambridge, MA, USA Mary S. Morrison, Christopher J. Podracky & David R. Liu
- Department of Molecular and Cellular Biology, Harvard University, Cambridge, MA, USA Mary S. Morrison
- Department of Chemistry and Chemical Biology, Harvard University, Cambridge, MA, USA Mary S. Morrison, Christopher J. Podracky & David R. Liu
- Mary S. Morrison